SUCCESS
- Michael Hochberg
- Apr 9
- 26 min read
We often associate success with winning, but more fundamentally success is persisting. We see success in seemingly endless physical and chemical structures, the marvelous diversity of life in, on and above Earth’s surface and of course in the humans that inhabit and have transformed the planet and its biosphere. Success is central to everything.
Understanding the world of us and around us is only possible if we do a deep-dive into this thing of success. As I will explain through physical and biological examples there is more here than meets the eye, the most amazing fact being that given the right conditions success is inevitable. Many will disagree, but given my definition of success I’ve just proved it to you! No less amazing, success by its very nature inevitably generates challenges – every silver lining hides a cloud – and even if you are not thinking about the wonders of success, you probably are (or will be) about its consequences, the most preoccupying being daily reminders of existential threats, which will remain nameless until later essays in this series. Just so you know, I don’t have magic solutions to fake news or climate change, to name but two pervasive threats, and for that matter nor do any of the countless people who have written books about the good and the bad on Earth and what humans can, should or must do to make things better. Much of what has been said although insightful is no more than hopeful and – worse – unimplementable. Rather, I advocate deep understanding and not knee-jerk panic and reactive planning. The theory of success is multi-level, that is it encompasses the microscopic, mesoscopic and macroscopic phenomena of success, and it considers bottom-up, top-down and everything in-between approaches. These provide a framework and a compass for pin-pointing problems, why they are problems, and startling conclusions about the common nature of all problems. Clearly an ambitious proposition! Stay with me and you will see how and why it works.
Defining life
I’m fortunate to be an external faculty member at the Santa Fe Institute. SFI is a think-tank and a very special one at that. They study complexity in all of its guises from understanding time and aging, to how life is the organization of energy and information, to predicting the outcomes of democratic elections. SFI is unique in viewing systems – physio-chemical, biological or artificial – as manifesting from fundamental laws and understandable from general theories. The interdisciplinary nature of the institute means that when you bump into anyone at tea and talk about an idea, observation or an unsolved question, you are almost certain to get a different perspective from your own. Feeling challenged in this way can be a bit disarming, but when discussions are carried through you come away changed.
Different meetings have different dynamics. Tea talks have their own spin – they tend to be brief – usually no more than 30 minutes. You join or leave when you wish. Occasionally there is a rare special spark that leads to more discussion and perhaps a collaboration leading to a scientific publication down the line. Office conversations on the other hand usually being à huis clos are a very different beast. To this, each summer when I visit SFI I have a new office mate; sometimes a resident professor, others another member of the external faculty. Entering my office upon arrival at SFI is therefore always a mixture of anticipation and excitement to see who this person will be. Invariably a conversation is struck and we probe each other intellectually to zero-in on common ground (and often there is). A one-on-one office conversation with someone you have never met before is a special creature. It has the power to generate unexpected ideas. As visitors do not choose who they room with one may find oneself sharing the office with a physicist, archeologist, neurobiologist, an economist or someone sufficiently interdisciplinary to call themself a complexity scientist!
I shared an office in April 2019 with Michael Lachmann, a resident faculty member at SFI. Our academic backgrounds overlapped but were sufficiently different to make for lively and enriching conversation. As per the way conversations begin the first topic was “what are you working on at the moment?”. Michael gave a few key phrases of his current projects. One of them stood out. Life. ‘What is life?’ ‘What is living?’ Not a minor topic! and one that would interest anyone immensely, not only because many biologists hold these questions on perpetual standby but also since I had a pet project to write an essay on this very topic. We discussed life in some detail, sometimes agreed, often disagreed, and – at least speaking for myself – found my entrenched views changed after a mere half-hour.
Lachmann’s idea – published in Aeon in collaboration with Sara Walker – is simple and gets to the nub of differences between life and living. Life is an evolving lineage. Both humans and sofas are life since both can be traced backwards in time to their ancestors and ancestral states. This is important because it says life always has a progenitor at least one step back and that complexity and diversity might emerge through reproduction and the generation of and selection on variants. Lachmann and Walker argue (Lachmann and Walker 2019) that what most people would not consider life or alive – for example, the lineage of sofas (yes, what we sit or lay on) – has gone through considerable modification and diversification but can be traced to humans and their lineage. Sofas cannot reproduce and modify by themselves (at least not yet). Alive things – specifically humans – do this for them. Erwin Schrödinger in his book What is Life? argued that being alive is “having the capacity to use free energy to maintain negative entropy in structure and function, so as to survive and reproduce” (Schrödinger 1992). Certainly a mouth full, but the crux of being alive is locally beating the second law of thermodynamics, that is generating structure and function counteracting their decay. Alive things acquire, interpret, triage, encode, store and recall information about the world. Thus, humans are both life and alive. Sofas are just life because they have no intrinsic mechanisms to generate and maintain their structure and function.
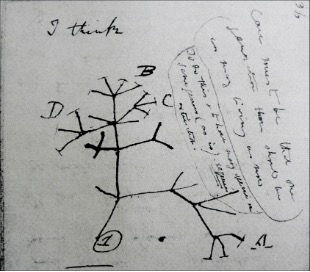

Figure 1. (Left) Darwin’s representation of speciation through time showing clades A-D and back to a common ancestor labelled 1. (Right) Analogous in some ways to biological speciation, technological innovation can stem from improvement on current phenotypes (Hochberg et al 2017). Shown here is the course of innovations in horn valve design (Tempkin & Eldridge 2007).
Lachman and Walker’s ideas are useful in conceptualizing, comparing and contrasting life and living, but many fundamental questions remain. Does life have a single ancestor or does it gradually emerge from a fuzzy network of semi-living and non-living progenitors? Can’t the fight against entropy associated with being alive extend to non-alive entities characterized by life phylogenies? That is, for example, sofa condition (upholstery, structure) can be maintained by sofa homeostats, that is, humans. Of course, in any form of homeostasis work is done and entropy (disorder, decrease in useful energy) increased overall. But if we assume that artificial and designed life can be functionally-dependent on human users then it is not too farfetched to regard sofas, computers and cars as somewhat alive. This will be touched on below and become important in subsequent essays in this series.
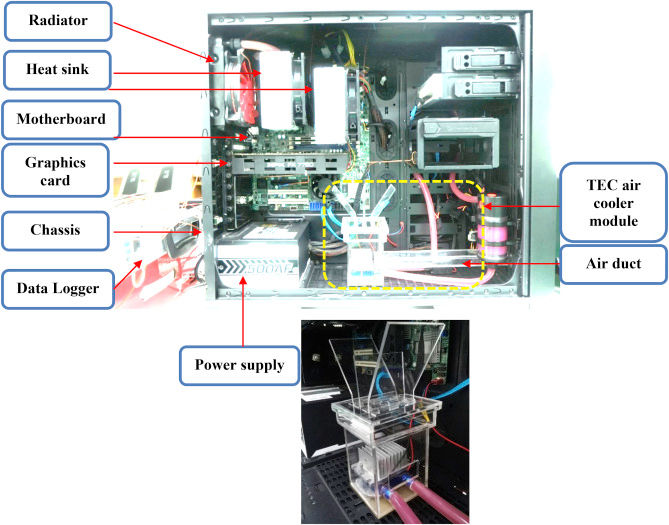
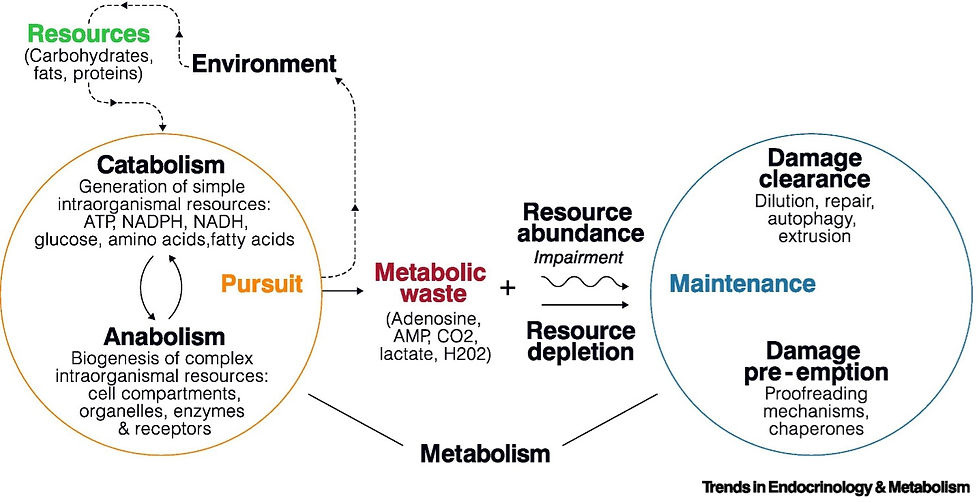
Figure 2. Energy processing and entropy in (left) a biological cell (Moldakozhayev and Gladyshev 2023) and (right) a personal computer (Wiriyasart, Hommalee, and Naphon 2019).
The splendor of these and other classifiable objects having function – that is, the object imparts information of some kind – comes closer to what we usually regard as being alive. Different models of sofas, computers or cars are “species” of a sort. Of course they do not survive and reproduce on their own, but that does not take away from the fact that they are birthed, survive, maintained, and eventually perish. They are also submitted to selection since their very existence and coexistence with, or replacement by, new variants depend on humans: technological capacities, market preferences and even arbitrariness or whimsy. How do these and other life-like species grow and spread? Why do others stagnate and eventually go extinct? Why do some species change rapidly whereas others evolve slowly or not at all? Said like this it’s difficult to know whether we are talking about butterflies or kitchen sinks. As it turns out we are talking about both.
Evolution and maintaining order are key to what will follow and as disparate as the two may seem, they find unification in central notion of Success. Success is how a system – an object, an individual, a collective, a population, a species, a community – persists, and to persist, it must grow and adapt.
Success = persisting, growing, adapting
Taken at face value, success is incredibly boring! It comes down to doing the best possible given circumstances. But success turns out to have a slant that makes it anything but boring – this slant explains why we are here and indications of where we are going. Exaggeration?? Not at all. With some unpacking success will be understood in all of its beauty… and tragedy.
To get a first glimpse at success as a prelude of what it means to life and being alive let’s examine perhaps the closest thing without actually being either: Crystals.
Crystals
Like many curious kids of pre-Internet generations, I had a rock collection. I was attracted to color and pattern – anything out of the ordinary. Most of my specimens had one or more of bright colors, metallic flecks or beautiful geometric shapes. One piece stood out from the rest. It was a flat (1 cm), multi-peaked pink mineral composed of perhaps two dozen closely packed crystals on a bleached-white, salt-like glistening bed, the full piece being about 5 cm by 3 cm. I found it when I was 11 or 12 years old while prospecting on the banks of a tributary of the Los Angeles River, not more than 100 meters from my house. I never really wondered how physics, chemistry and simple molecules must have interacted over unimaginably long periods in the formation of this structure (and original parts of it that were surely scattered elsewhere), nor the tectonics that could have transported it to the surface, no less how it might have been discovered multiple times over the many thousands of years of human habitation in what is now called the San Fernando Valley.
There was something about this crystal that made it different from the other pretty rocks I’d collected and it was only in a middle school science class that part of the answer was revealed. That day’s lecture was about the formation of crystals. The teacher clarified that although all inorganic crystals are derived from minerals many crystal types could only be obtained under unimaginably extreme and specific conditions. He passed around stunning specimens of cubic galena and pyrite. I was struck by their other-worldliness: The uniqueness of their color and amazing simplicity and regularity in their geometry. I thought of the translucent pink tetrahedral shapes of my crystal back home. It was as if these different entities were cousins at some fundamental level.
***
Geologists, naturalists, physicists and philosophers have long viewed crystals as life-like curiosities, but finally not fulfilling the minimal requirements for life. Or rather, more accurately fulfilling a characteristic of life-like non-life, that being non-hereditary emergence.
The fantastic naturalist that was Charles Darwin extended well beyond his contributions to the study of biological evolution. Darwin was an ardent geologist. When delving into Darwin’s own notes we often cite his famous Red Notebook B where he drew a representation of a phylogeny on what would become his theory of evolution by natural selection. But what about Red Notebook A? Did it have pages as transformatory as the iconic “I think” in Notebook B? Well indeed, Darwin being a student of contemporary geology and physics hypothesized that geological and gravitational forces interacted to turn basalt into crystals deep in the earth as revealed by the expulsions of volcanos. So even if not as Earth-shattering as his theory of evolution by natural selection Darwin’s ideas on crystal formation reveal that he was fundamentally interested in laws of both the biological and the physical world. Laws turn out to be important in what follows.
By no means did crystal wonders start or stop with Darwin. Shortly after Darwin, German zoologist Ernst Haeckel marveled at the life-like properties of crystals (Sunagawa 1990) that thanks to differences in environmental conditions, can show considerable diversity in composition and form. There are approximately 4000 ‘species’ of mineral and no two natural crystals are ever identical. Haeckel viewed them as akin to individuals but also likened crystals to dead bodies. He was particularly fascinated by liquid crystals – mesophases found in living organisms – which he viewed as structures uncannily biologic-like. He observed many phenomena in liquid crystals that were suggestive of being alive such as growth, ‘copulation’ and regeneration (Geelhaar, Griesar, and Reckmann 2013). Haeckel’s observations were a prelude to a much more important idea: The centrality of crystalline structures in living creatures. So called ‘biogenic crystals’ pervade biology with examples including pigments and the crystalline lens in animal eyes (Prywer 2022). The regularity and stability of crystalline structures may have been associated with – and even responsible for – success in the earliest life-forms.
Crystals catch the attention of the curious and the list of luminaries who either dabbled with or did serious observation and research on crystals includes Isaac Newton (lead glass diffraction), Louis Pasteur (asymmetric crystals) and Linus Pauling (a-helix). Add to this none other than the physicist Erwin Schrödinger, better known for his simultaneously dead-alive cat which codified the central idea of quantum physics. Schrödinger reframed the idea of hereditary information made previously by geneticist Herman J. Müller in what Schrödinger called the "aperiodic crystal" in his 1944 book What is Life? The Physical Aspect of the Living Cell (Schrödinger 1992). Schrödinger’s exposé is as close as any to likening crystals to life by describing how information as hereditary material is stored in molecular form. Information sufficient for life is by definition neither too repetitive (ordered = simple) nor random (disordered = complicated). The fact that some inorganic crystals are at the repetitive boundaries of this description is part of the basis for theorizing them as precursors to life.
In their wonder of crystals these giants were on to something essential about success. In a nutshell: All systems have boundaries. The crystals we find on Earth’s surface can become more massive than any human being but don’t and can’t grow forever. It’s not for want of more space – crystal formation requires specific physical and chemical conditions and in particular the raw material upon which the crystal is derived. For example, the largest crystalline structure on the planet Earth is actually inside it. Earth’s inner core is composed of a special hexagonal close-packed form of iron called ε-iron. The inner core is quite distinct from the outer core (also largely composed of iron) and is remarkably different in structure and dynamics to the Earth’s onion-like outer layers. This is because environmental physics (temperature, pressure, gravity, magnetism) of the crystalline core – even if highly dynamic – are remarkably stable. Earth’s crystalline core may be much younger than the planet itself, recent estimates putting this at approximately 550 million years (Bono et al. 2019). If all this sounds very “so-what” then consider the following: The magnetic field generated by Earth’s inner molten iron core is thought to shield the Earth’s surface from harmful radiation (Zhou et al. 2022) and enable not only the persistence of otherwise doomed life but have contributed indirectly to the Cambrian Explosion itself – and ultimately, way down the line, us!
***
Crystal emergence is eerily life-like. A crystal grows from a seed – so called nucleation – or from a disturbance generating seeds. Even if under certain circumstances crystals can promote their own growth (De Yoreo and Vekilov 2003) they don’t transmit their crystalline state willy-nilly à la Ice-nine in Kurt Vonnegut’s Cat’s Cradle. This said, if water is supercooled below 0°C and somehow disturbed (by a shock or introduction of a particle) then an array of ice crystals will rapidly form and spread. The growing crystal piggy-backs on its most recent self. The crystal will continue growing as long as the necessary conditions are met along the structural front (Hartman 1964). One can almost equate crystal growth with biological growth. Crystals in fast motion do ‘grow’ (you will know this if you have ever grown salt crystals) and can appear to make more of themselves, but what we observe with the naked eye are just macro-scale patterns of what are in fact the finer-scale processes of physics and chemistry. Physical laws do not mutate but rather manifest depending on the surrounding environment including temperature and specific building blocks – atoms or molecules comprising the crystal (Earle 2019). Thus akin to a biological organism, growing crystals transfer energy and consume resources. But unlike biological life, inorganic crystals are just physio-chemical reactions playing out, with few steps between what they eat and what they are.
The relative simplicity of crystal formation is not cause for striking them completely from the Pantheon of being on the edge of alive. Although crystals clunk up some of the steps, their downfall is that they are completely dependent on molecular aggregate stability and on their surroundings. They are passive, or rather, reactive. They can’t repair themselves when damaged or when environments go wrong. Their inevitable erosion over sufficiently long time periods means that despite their ubiquity the vast majority of the crystals we see in the Earth’s upper outer crust are (very, very) tiny. Just examine a few grains of sand with a magnifying glass or a speck of dirt under a microscope to see the crystal status quo.
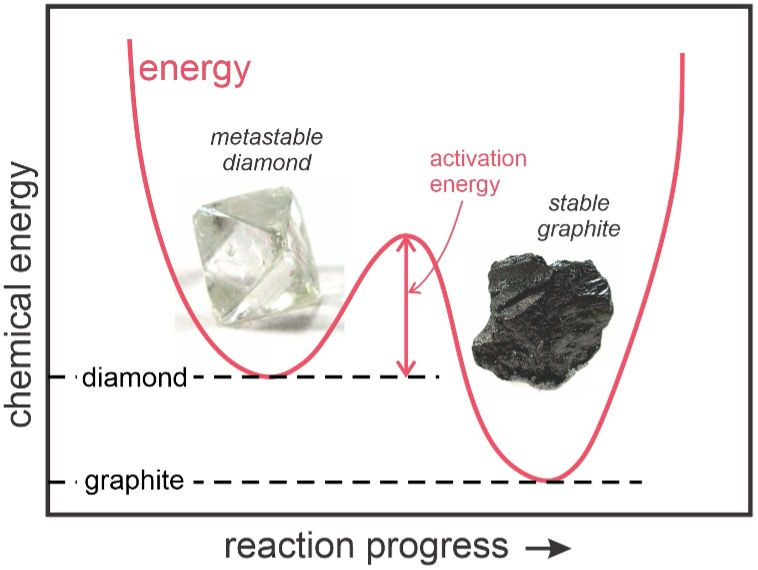
Figure 3. Carbon in two crystalline states showing the activation energy needed for transformation from diamond to graphite. Caption: opengeology.org/petrology/wp-content/uploads/Ch11/diamond-and-graphite.
What about crystals as life? If we accept the definition put forward by Lachmann and Walker then some crystals at least come close. The Earth’s inner-mantle conditions have been a breeder for crystal structures over the past 4+ billion years (Earle 2019) as evidenced by the prevalence of crystals in the Earth’s upper crust. The Bowen reaction series (Bowen 1922) in particular has generated fascinating lineages of different mineral crystals over enormous lapses of time through the cooling of the Earth’s magma in conjunction with key reactions involving silicon dioxide, calcium and sodium. Despite the fact that this is a lineage there is no true reproduction occurring. Well, sort of… There actually is reproduction of sorts. At least in theory (Davey 2004) and in the laboratory (Anwar, Khan, and Lindfors 2015), nucleation and in particular seeding – or “secondary nucleation” as it is called – can act as a crystal breeding factory. Secondary nucleation can spit-out baby crystals at impressive rates. Whether such crystal breeding actually happens in nature is unknown.
But the story has more wrinkles. In 1966 Cairns-Smith published a very compelling theory building on Bernal’s 1951 classic book The Physical Basis of Life claiming that life started with silicate crystals in the form of clay (Cairns-Smith 1966). The basic idea is that under certain conditions clay can auto-catalytically produce more clay (through crystallization and “layer cleavage”) and might have provided a basis for the first organic replicators. Thus, ionic interactions at the clay surface replicate charges and store them. This provides a template for organic molecules to enter the system and if conditions are right take it to the next level of pre-life. That’s amazing in itself, but Cairns-Smith pushed further arguing for how silicate crystals are (almost) alive! According to him the physical dislocation and chemical imperfections in crystals can be propagated – not quite Darwinian mutations but rather a sort of Lamarckian process whereby genotype and phenotype are one and the same. Nevertheless Cairns-Smith actually did envisage this as a sort of Darwinian process – clays can perform both functions of DNA and of proteins – and referred to the pattern in imperfection variation as a genograph. He was in some respects prescient of later work on life in suggesting that crystal growth and evolution required static disorder but dynamic order.
Reading Cairns-Smith’s paper today one is struck by his scientific honesty in outlining just how specific conditions might need to be in order to get the clay replicative machinery going. This said, he then goes to great length to sketch scenarios for clays as the first genome-like replicators and organisms and finally veers off the rails in suggesting that clays were the first viruses to infect the Earth. Despite the liberties he takes the basic idea and mechanism are data-based. Indeed Lionel Penrose (father of the famed Nobel Prize physicist, Roger Penrose) provided a simple mechanism for how machines of different sorts could replicate using inorganic crystals and organic molecules such as polysaccharides, amino acids, and phosphates as potential polymerization candidates (Penrose 1959). Penrose’s theory and mechanism will figure in a later essay in this series but coming full circle in the present, Cairns-Smith conceded that the clay proto-organism is at the mercy of the environment and in fact whereas clay – specifically micaceous clay – could have contributed to biopolymers of the first organic life there is no rigorous demonstration that it itself was pre-life (Hansma 2022; Hazen and Sverjensky 2010). The only processes intrinsic to crystals putting them at the doorstep of life or alive are nucleation and cleavage, the latter which are planes of weak bonds that are sources of crystal splitting.
As semi-scientific as are the above claims, we can’t completely rule-out crystals as being something akin to life. After all their mineral sources fall into a finite number of species-like classes. So let’s go back once again to why minerals and their crystal phenotypes are at least on the edge of life and alive. I’m getting a bit ahead of myself because this phenomenon makes its full entry in a future essay, but this is the place to start. Take variation, the very commodity required for evolution via natural selection and the generation of new genotypes, species or lineages. Minerals do indeed show variation in shape and color and even within a crystal aggregate there can be subtle variations in these. Again let’s not get carried away: It’s the laws of physics and chemistry in the context of local environmental conditions that produce characteristics bordering on life, but not quite enough to be life.
My conclusion is that crystals live happily at the fringes of life and being alive. They grow. Some live fast and die young – such as snowflakes landing on a tongue – whereas others grow old without showing any signs of aging – such as the diamond cubic that can persist unchanged for millions if not billions of years. The take-home message is not so much the spectacular range of mineral species and geometric crystal forms – yes, they are amazing – but rather that the fact we observe them is thanks to their stability. Under hypothetical unchanging optimal conditions, crystals are forever, but in the dynamic natural world they are at the mercy of the laws of physics and the vagaries of the environment. Crystals know nothing akin to biological metabolism and maintaining it through homeostasis and defense. Crystals grow and are beautiful, but alas they are dead.
H2O
The most familiar substance in our lives is water, and the water molecule, despite being stubbornly stable, is the antithesis of stability when it comes to being in crowds, be it liquid, gas or solid. Our foray into the wonder of water begins with a true story that – as a young scientific researcher – blew my mind.
Of the many research articles that gained both scientific and media attention in the 1980s one in particular stood out, not because of its wonder, but rather because the findings were theoretically impossible. The very unassuming title “Human basophil degranulation triggered by very dilute antiserum against IgE” published in Nature magazine was notable for having an Editorial Reservation by the journal editors at the end of the article. The study led by Jacques Benveniste (Davenas et al. 1988) implied that homeopathy had a mechanistic molecular basis. Homeopathy is the diluting of an ‘active’ agent to the point where the agent is either at infinitesimal concentrations or entirely absent. The only way that homeopathy with (near) zero active substance could work based on a molecular mechanism is either if the dilutant maintains some kind of memory of the active agent, if the agent results in an autocatalytic effect at its target, or if the dilution procedure is faulty such that the active ingredient remains present. Regardless, science did and still resoundingly reject any mechanistic basis for homeopathy. To the extent that positive effects of homeopathic treatments are based on objective measures this would suggest a placebo effect (which is not a problem in itself!) or a conjuncture effect, that is the treatment coincides with the positive effect of other, independent mechanisms (such as the immune system). The Nature study did not investigate the mechanism behind the result and so was unscrupulously taken up by some of the media as a scientific basis for homeopathy.
The critical reactions by the scientific community prompted an investigation by Nature magazine. After all, they published the study based on peer review and editorial oversight. That this was not a straightforward process was indicated by the end-of-article editor disclaimer. Yet the journal could not anticipate whether reasons beyond the declared scientific procedure explained the findings – that is, whether some form of unintentional bias, hidden mechanism, or intentional tampering occurred. Ultimately, investigation by Nature magazine of Benveniste’s lab concluded an experimenter effect. Thus one possibility to explain the original study’s significant results is that dilutions were imperfect and the active molecule was present at appreciable levels even though according to the dilution levels it should have been disappearingly low or absent. So insufficient rigor in scientific methods could explain the results. This follow-up called into question the study’s findings and conclusions (Maddox 1988).
What seems most surprising in the Benveniste affair is that the authors did not seem to question their results either in the original article nor in the years’ long discussions that ensued. After all the study implied that water molecules could retain the memory of past interactions with an active molecule. If true, this could overturn certain laws of physics and chemistry. Of course it was not true, which should have set-off bells and whistles either before or after peer review at Nature. Following publication of the study, physicists had a go at estimating how long water holds memory after a disturbance and although this depends on conditions, calculations put the memory time on the order of pico-seconds (Laage et al. 2011) – that is one-thousandth of a billionth of a second! At the lab temperature of Benveniste’s experiments the water molecule is hyper-stable.
Yet there is a catch. One could imagine that water retains memory in another way. Although its molecular configuration doesn’t change, somehow the aggregate of molecules orients so as to maintain the imprint of the now long-gone additive. Despite continued interest and even hope by some that water might maintain memory in some (any) way – still an attractive subject in the journal Homeopathy – physics shows this is wrong, at least for pure H2O. Like the blisteringly fast snap-back of each individual water molecule groups of H2O fill inter-molecular space with lightning-fast speed and ‘rub shoulders’, constantly wiggling and traveling past one another. So the catch is caught.
Despite the impossibility for pure H2O molecules in liquid phase to keep memory in any useful way, at entry into gaseous phase what-goes-where is not completely random over micro-spatial and temporal scales. This is in fact analogous to water coming off of ice just above 0°C. As such, ice, water near 0°C and water vapor near 100° are objects in a sense but you would not be able to differentiate one collection of H2O molecules from another in any of these phases based on the spatial arrangement of component molecules. Even if you gave each and every H2O molecule in a drop of water a unique spatial identifier, in less than a second the arrangement would become unpredictably different. Add to this the thermal dynamics with surroundings and it becomes apparent that physics, chemistry and environment intermingle in order and disorder. As we will see later, physics, chemistry and environment also play roles in the order and disorder of biological life itself.
This is all simple, clean and tidy. Sure, we can forget the hustle and bustle of liquid and gas and focus on the stability of ice. But if you have ever seen a demonstration of how experts determine the risk of an avalanche then you know that ice crystals at scales of about 1 millimeter can be lumped into different categories associated with the probability of snow- or ice-pack slippage. This is very important if you are on or near a mountain side with a slope around 38°, which is the sweet-spot for many avalanches. Though outside of avalanches macro-scale ice instability is not very important in every-day life. Well consider the exciting, but unimportant microscopic world of ice. When we bore-down to the molecular scale it turns out that there are at least TWENTY possible crystalline phases of ice (Hansen 2021). The one we all know – and indeed the only not requiring extreme low temperature and high-pressure conditions – is called hexagonal ice or Ih.
The different crystalline forms of water reflect positioning in space, regularity of the crystal and bending of the H2O molecule itself. Some can only be obtained by playing laboratory tricks, others are metastable (that is stable as long as conditions are right) and yet others are irregular. Some only occur at remarkably precise temperature and pressure conditions. Different forms can coexist within the same crystal. Linus Pauling, the polymath whose contributions to chemistry earned him a Nobel Prize in 1954 made the startling discovery using X-ray diffraction that Ih is not only irregular but ‘dynamic’ (there is residual entropy in the system caused by molecular reorientation at temperatures above about -70°C) (Pauling 1935). Ih shows disorder, which is a consequence of so-called “ice rules” developed two years previous to Pauling’s paper by Bernal and Fowler. The basic idea is that the intermolecular forces exerted by the two-proton state of oxygen are insufficient to stabilize the crystal and as a result at the low temperature limit, conformations are disordered and dynamic. Despite this randomness Ih is a crystal state. As temperature is dropped and pressure increased ice tends to become denser, ordered (loses entropy) and conform to either a stable or metastable (a local but not global equilibrium state) crystal. Alas, outside the laboratory zoo, except for the strange Isd (with stacking disorder of cubic (Ic) and horizontal (Ih) motifs) you are unlikely to see any of the 20-or-so I-forms simply because the conditions they require are not (easily) found on our planet.
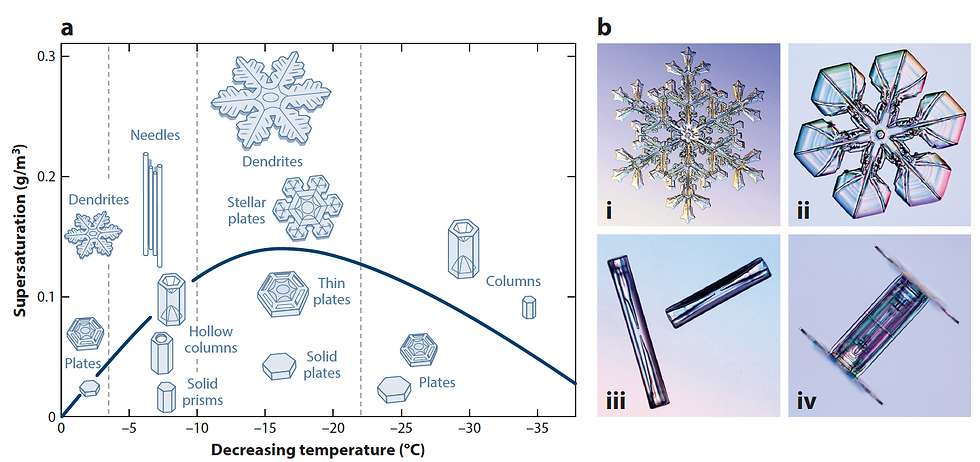
Figure 4. (a) Dependence of snow crystal growth on temperature and H2O saturation. The line shows the supersaturation of liquid H2O relative to ice. (b) Examples of snow crystals at -15°C (i, ii), -5°C (iii) and a hybrid crystal (iv). (Libbrecht 2017).
But let’s stick with the far-from-mundane Ih. We know how physical conditions such as temperature and pressure can change ice crystal structure. But ice crystals hide a secret in plain sight. Molecular ice at Earth temperatures is somewhat unstructured and dynamic. This may very well contribute to explaining the most recognizable H2O crystal form – the snowflake: No two snowflakes are exactly the same. And the same kaleidoscopic property could apply to other inorganic crystals on Earth.
Each snowflake emerges from a unique de novo sequence of events. A frozen accident that does not propagate beyond the flake. As such even if different classes of snowflake shapes can be assigned to quasi-species snowflakes have no lineage among ice crystals. Infinite snowflake diversity is just physical laws playing out in the unique microenvironment of each growing ice crystal – micro-thermal fluctuations, micro differences in air pressure, microscopic interactions with gases including H2O and micro particles with which the H2O forms what amounts to an individual one-way-dissipating system. Freezing water vapor can produce snowflake variation à go-go despite rock-hard determinism in the fundamental laws of physics. No mutation to the laws means no deleterious baggage and persistence – that is success – for the individual snowflake as long as environmental conditions are right. But as for other crystals the snowflake version of success – rigid stability – is limited. Snowflakes eventually stop growing and don’t make baby snowflakes. Like ice more generally, they are a temporary frozen event, either decaying through sublimation to gas, thawing to liquid, or remaining inert – that is frozen in time. So the tragedy for snowflakes is that their stable, stupendous, beautiful variation means little to their persistence. Their success is transient.
Persistence is not forever
Perfect stability is nothing but inertness. Diamonds may be forever but once shaped by humans, although these carbon crystals may adorn museums or people they otherwise go nowhere. As mesmerizing as they are, diamonds, other gemstones and for that matter any inorganic crystal one finds in a display case can’t grow let alone give birth to new crystals. Even if imperceptible on human time scales crystals invariably fissure, break and erode due to pressure and friction. Saving this, crystals structurally change over human, geological or cosmic time scales depending on temperature, pressure and the entropy landscape of the mineral. A crystal can be reconfigured to a higher or lower entropy state. In the case of carbon, the higher entropic / lower energy state is graphite – aka pencil lead. At the ambient conditions the natural transition from diamond to graphite has been speculated to take on the order of billions of years (Baker 2018).
Overdrive as a (temporary) adaptation
We might therefore be led to the conclusion that change – possibly lots of it – is a requirement for success. True, frequent phenotypic change in some entities is crucial to survival, a well-known example being immune system evasion through antigenic variation in HIV, the virus associated with AIDS. Less well known are bacteria that mutate at higher than usual rates, so-called ‘hypermutators’. Hypermutators of the pathogenic bacterium Pseudomonas aeruginosa, responsible for pulmonary infections and in particular those in people with Cystic Fibrosis, can mutate orders of magnitude higher than the usual rate. This enables mutator strains to quickly adapt to lung environments and specifically in those patients receiving antibiotic treatments (Oliver et al. 2000). Although important to microbial populations faced with novel or changing environments, high mutation rates – particularly in long-lived species producing few offspring, such as humans – is generally bad. Microbial species under stress benefit from it because their populations are often enormous and a very small proportion of lucky individuals will win the mutation lottery, deal better with challenging environments, and prevail over those unable to cope with the stress. But although this may be prevalent in the microscopic world of microbes, it is certainly not the rule in species we can see with the naked eye. Many if not the majority of macroscopic species on our planet have found a general solution to coping with challenging environments: They are plastic. Plasticity enables change when confronted with novel situations. So as long as environments do not change enough to endanger stability it is best to have a phenotype that itself does not change. When environments are prone to venture out of the object’s comfort zone then the capacity for change – either plasticity, mutation or recombination – becomes important if not essential.
Punchline
We now have an embryonic framework for a theory of success. The astonishing conclusion is that despite stability and persistence, successful species are never perfect. Crystal-success is inertness and inability to adapt. Crystals are sitting ducks. Biological-success on the other hand is always tracking, but nevertheless being one or more steps behind the environment (Wiser, Ribeck, and Lenski 2013)(Adams et al. 2017).
Success is Goldilocks stability. Too much and the thing is merely a reflection of physio-chemical forces tickled by environments and ultimately doomed to dwindle and die. Too little and it’s not itself for long and inevitably destined to burn out. Just right and the species grows, adapts, persists and ultimately evolves.
Imperfection is a hallmark of success
Imperfection drives natural selection and selection, in turn, ups the ante on adaptation. Adaptation is never perfect since systems can always improve especially when faced with a changing environment. What could possibly be more simple and beautiful? In this light the adage success breeds success begins to make sense. To see why once started success is unstoppable we need to take a deep dive into its drivers, starting with how and why life grows. Stay Tuned.
References
Adams, Alyssa, Hector Zenil, Paul C. W. Davies, and Sara Imari Walker. 2017. “Formal Definitions of Unbounded Evolution and Innovation Reveal Universal Mechanisms for Open-Ended Evolution in Dynamical Systems.” Scientific Reports 7 (1): 997. https://doi.org/10.1038/s41598-017-00810-8.
Anwar, Jamshed, Shahzeb Khan, and Lennart Lindfors. 2015. “Secondary Crystal Nucleation: Nuclei Breeding Factory Uncovered.” Angewandte Chemie International Edition 54 (49): 14681–84. https://doi.org/10.1002/anie.201501216.
Baker, I. 2018. “Diamond. In: Fifty Materials That Make the World.” Springer, Cham.
Bono, Richard K., John A. Tarduno, Francis Nimmo, and Rory D. Cottrell. 2019. “Young Inner Core Inferred from Ediacaran Ultra-Low Geomagnetic Field Intensity.” Nature Geoscience 12 (2): 143–47. https://doi.org/10.1038/s41561-018-0288-0.
Bowen, N. L. 1922. “The Reaction Principle in Petrogenesis.” The Journal of Geology 30 (3): 177–98. https://doi.org/10.1086/622871.
Cairns-Smith, A.G. 1966. “The Origin of Life and the Nature of the Primitive Gene.” Journal of Theoretical Biology 10 (1): 53–88. https://doi.org/10.1016/0022-5193(66)90178-0.
Davenas, E., F. Beauvais, J. Amara, M. Oberbaum, B. Robinzon, A. Miadonnai, A. Tedeschi, et al. 1988. “Human Basophil Degranulation Triggered by Very Dilute Antiserum against IgE.” Nature 333 (6176): 816–18. https://doi.org/10.1038/333816a0.
Davey, Roger .J. 2004. “How Come You Look so Good?” Nature 428 (6981): 374–75.
De Yoreo, James J., and Peter G. Vekilov. 2003. “3. Principles of Crystal Nucleation and Growth.” In Biomineralization, edited by Patricia M. Dove, James J. De Yoreo, and Steve Weiner, 57–94. De Gruyter. https://doi.org/10.1515/9781501509346-008.
Earle, Steven. 2019. Physical Geology, 3.3 Crystallization of Magma. 2nd edition. Victoria, B.C.: BCcampus, BC Open Textbook Project.
Geelhaar, Thomas, Klaus Griesar, and Bernd Reckmann. 2013. “125 Years of Liquid Crystals-A Scientific Revolution in the Home.” Angewandte Chemie International Edition 52 (34): 8798–8809. https://doi.org/10.1002/anie.201301457.
Hansen, Thomas C. 2021. “The Everlasting Hunt for New Ice Phases.” Nature Communications 12 (1): 3161. https://doi.org/10.1038/s41467-021-23403-6.
Hansma, Helen Greenwood. 2022. “DNA and the Origins of Life in Micaceous Clay.” Biophysical Journal 121 (24): 4867–73. https://doi.org/10.1016/j.bpj.2022.08.032.
Hartman, P. 1964. “Structure, Growth and Morphology of Crystals.” Zeitschrift Für Kristallographie - Crystalline Materials 119 (1–6): 65–78. https://doi.org/10.1524/zkri.1964.119.16.65.
Hazen, R. M., and D. A. Sverjensky. 2010. “Mineral Surfaces, Geochemical Complexities, and the Origins of Life.” Cold Spring Harbor Perspectives in Biology 2 (5): a002162–a002162. https://doi.org/10.1101/cshperspect.a002162.
Laage, Damien, Guillaume Stirnemann, Fabio Sterpone, Rossend Rey, and James T. Hynes. 2011. “Reorientation and Allied Dynamics in Water and Aqueous Solutions.” Annual Review of Physical Chemistry 62 (1): 395–416. https://doi.org/10.1146/annurev.physchem.012809.103503.
Lachmann, M., and S. Walker. 2019. “Life ≠ Alive.” Aeon, June 24, 2019. https://aeon.co/essays/what-can-schrodingers-cat-say-about-3d-printers-on-mars.
Maddox, John. 1988. “Waves Caused by Extreme Dilution.” Nature 335 (6193): 760–63. https://doi.org/10.1038/335760a0.
Moldakozhayev, Alibek, and Vadim N. Gladyshev. 2023. “Metabolism, Homeostasis, and Aging.” Trends in Endocrinology & Metabolism 34 (3): 158–69. https://doi.org/10.1016/j.tem.2023.01.003.
Oliver, Antonio, Rafael Cantón, Pilar Campo, Fernando Baquero, and Jesús Blázquez. 2000. “High Frequency of Hypermutable Pseudomonas Aeruginosa in Cystic Fibrosis Lung Infection.” Science 288 (5469): 1251–53. https://doi.org/10.1126/science.288.5469.1251.
Pauling, Linus. 1935. “The Structure and Entropy of Ice and of Other Crystals with Some Randomness of Atomic Arrangement.” Journal of the American Chemical Society 57 (12): 2680–84. https://doi.org/10.1021/ja01315a102.
Penrose, L.S. 1959. “Self-Reproducing Machines.” Scientific American 200 (6): 105–14.
Prywer, Jolanta. 2022. “The Fascinating World of Biogenic Crystals.” Science 376 (6590): 240–41. https://doi.org/10.1126/science.abo2781.
Schrödinger, Erwin. 1992. What Is Life? The Physical Aspect of the Living Cell ; with, Mind and Matter ; & Autobiographical Sketches. Cambridge ; New York: Cambridge University Press.
Sunagawa, Ichiro. 1990. “Growth and Morphology of Diamond Crystals under Stable and Metastable Contitions.” Journal of Crystal Growth 99 (1–4): 1156–61. https://doi.org/10.1016/S0022-0248(08)80100-5.
Wiriyasart, Songkran, Chootichai Hommalee, and Paisarn Naphon. 2019. “Thermal Cooling Enhancement of Dual Processors Computer with Thermoelectric Air Cooler Module.” Case Studies in Thermal Engineering 14 (September):100445. https://doi.org/10.1016/j.csite.2019.100445.
Wiser, Michael J., Noah Ribeck, and Richard E. Lenski. 2013. “Long-Term Dynamics of Adaptation in Asexual Populations.” Science 342 (6164): 1364–67. https://doi.org/10.1126/science.1243357.
Zhou, Tinghong, John A. Tarduno, Francis Nimmo, Rory D. Cottrell, Richard K. Bono, Mauricio Ibanez-Mejia, Wentao Huang, et al. 2022. “Early Cambrian Renewal of the Geodynamo and the Origin of Inner Core Structure.” Nature Communications 13 (1): 4161. https://doi.org/10.1038/s41467-022-31677-7.
Comments